Circular 672
John Idowu and Sangu Angadi
College of Agricultural, Consumer and Environmental Sciences, New Mexico State University
Authors: Respectfully, Extension Agronomist, Department of Extension Plant Sciences; and Crop Physiologist, Agricultural Science Center at Clovis, New Mexico State University. (Print friendly PDF)
Introduction
Soil compaction occurs when soil particles are compressed together—especially when the soil is wet—destroying soil structure, reducing porosity, and leading to a more dense soil that is hard for crop roots and water to penetrate. Changes in agricultural practices, such as increased number of field operations and larger equipment, have made soil compaction more common on many fields. Field operations, such as silage crop harvest (Figure 1) when the soil is wet, can lead to severe soil compaction. Grazing cattle on range and farmlands is very common in the Southwest, but compaction due to grazing is short-lived due to freeze/thaw cycles, and the total weight of grazing animals is often not sufficient to initiate deeper compaction (Baumhardt et al., 2011). However, soil puddling (trampling of soil by animals under very wet conditions) can occur due to overgrazing, resulting in structural breakdown at the soil surface and subsequent crust formation when the soil dries out. Soil compaction affects many agricultural fields and can lead to yield reductions if not properly managed. Understanding soil's physical components will help you understand how compaction affects the soil.
Figure 1. Sorghum harvest in the field.
Soil Physical Components
Soil is made up of primary particles called sand, silt, and clay. These particles are classified by size according to the USDA textural classification (from largest to smallest): sand particles are 0.05—2 mm, silt particles are 0.002—0.05 mm, and clay particles are less than 0.002 mm. When arranged in soil, these particles have voids or pores between them, and the pores can be filled with either air or water. In well-balanced soil, the primary particles (sand, silt, and clay) occupy about 45% of the soil volume, while water and air together constitute about 50% of the soil volume. The remaining 5% is the organic component of the soil (Figure 2). The amount of the organic component is highly variable in the soil, depending on factors such as soil management, cropping systems, and climate.
Figure 2. General composition of soil constituents.
Soil Pores and Compaction
The pores or voids within the soil give it porosity. The pores in the soil have different sizes: large pores (macropores) have sizes >1 mm, intermediate pores (mesopores) are 0.01—1 mm, and small pores (micropores) are <0.01 mm (Luxmoore, 1981).
During a compaction event, the number and the distribution of pores within the soil is altered. Depending on the level of external force applied to the soil, compaction often leads to fewer large and medium pores and more smaller pores (Ankeny et al., 1990)
Macropores (large pores) are important for aeration and drainage of the soil. When rainfall occurs, water enters the soil and tends to fill all the pores depending on how much rain has fallen. When all the pores are filled with water, the soil is saturated. If large pores are deficient within the soil, the soil will stay saturated for a long period of time, and this can be harmful to plant roots because they need oxygen for growth. When the soil is saturated, more water cannot infiltrate the soil, and this can lead to an earlier and larger volume of runoff and soil loss due to erosion (Hanna and Al-Kaisi, 2009). With increased runoff, applied chemicals like fertilizers and herbicides are washed off from the field, which reduces the efficiency of these chemicals and contributes to environmental pollution. Additionally, a process called denitrification, which is the reduction of soil nitrates to nitrogen gas, becomes an issue in soils that stay saturated for very long periods. Denitrification has also been shown to lead to considerable nitrate losses from the soil and the emission of nitrous oxide, a very potent greenhouse gas (Yamulki and Jarvis, 2002). During compaction, these large pores are destroyed due to applied forces from farm equipment or farm traffic. This consequently leads to inadequate aeration and poor drainage of the soil.
Intermediate pores (mesopores) are important for short- and long-term moisture retention in the soil. Because of their smaller size, they are able to hold water in the soil against the force of gravity. The water can then be easily used by plant roots for several days after rainfall or irrigation events. The amount of these medium-sized pores in a soil will determine how long the soil can supply water to the plants between rainfall or irrigation events. With compaction, mesopores are destroyed and the water retention of soil is affected. Although compacted soil may still contain significant water, much of that water becomes unavailable as it is held very tightly in the smaller pores.
Types of Compaction
Soil compaction can happen at any layer in the soil and is classified into two major types—surface and subsurface—depending on where in the soil profile it occurs.
Surface compaction is when the dense soil layer occurs at the surface of the soil (Figure 3). This kind of soil hardening at the surface is also called soil crusting. Surface compaction happens when the surface soil aggregates are broken down through the impact of falling raindrops, runoff, standing water during irrigation, or tillage. Soil aggregates are the distinct arrangement of soil particles into "clumps" or "units," and these units are bound together more strongly than the adjacent soil. If the aggregates are broken down, the soil particles become rearranged into a thin, continuous, dense layer on the soil surface (Figure 4). When this thin layer dries up, a crust is formed uniformly across the soil surface, creating a compacted layer that restricts water and air entry and impedes seedling emergence (Morin et al., 1981). Impacts from the hooves of grazing animals (especially under overgrazing conditions) can also contribute to the breakdown of the surface soil aggregates and subsequent soil crusting.
Figure 3. Soil crust layer on the dry soil surface.
Figure 4. Water infiltration and runoff into well-aggregated and weakly aggregated soil.
The second type of compaction is subsurface compaction in which the compacted layer is found at a depth within the soil. This type of compaction may be natural or human-caused. Natural compaction occurs due to soil-forming processes and is normally found in the subsoil. Subsurface compaction can also be caused by modern agricultural practices such as tillage, use of heavy farm equipment, and farm traffic. For example, plowing the soil at the same depth for several years may cause a compacted layer just beneath the plow layer, which is called a "plow pan" (Figure 5). Compaction caused by farm operation can negatively affect the quality of the surface soil (0—12 inches) and make fields less productive for crop production.
Figure 5. Hardpan layer within the soil profile.
Damage Done by Compaction
Compaction caused by farm operations has been shown to affect the yield of field crops in several ways (Alblas et al., 1994; Arvidsson and Hakansson, 1991; Filipovic et al., 2006; Raghavan et al., 1978).
- Compaction creates a dense soil layer that is very hard for roots to penetrate. Roots tend to grow laterally when they hit this compaction zone. This severely limits the volume of soil that the roots can explore for water and nutrients.
- Compacted soil also limits the movement of water into the soil, thereby creating conditions that favor soil erosion and runoff. With much of the water running off, or being evaporated in the case of irrigated lands, the amount of water that goes into the soil and is made available for crop use is limited.
- Less water will be available in the soil for crop use due to the abundance of micropores that are holding the water tightly because of the destruction of medium and large pores by compaction.
Actual yield loss by compaction varies depending on crop type, growing environment, and any practices adopted to offset compaction. Some crops are known to penetrate hardpan better than others. If the crop is frequently irrigated or receives frequent rainfall, then root restriction by hardpan may be less detrimental.
How to Measure Soil Compaction
It is important for agricultural producers to manage their fields to minimize compaction. In fields where soil compaction exists, specific practices need to be carried out to ameliorate the compaction. This will guarantee better and more consistent yields across the field. The first step in managing soil compaction is to determine if a compaction problem exists. A simple way to observe compaction is to use a spade to dig up a section of the soil along with crop roots. Another simple observation is to pull out well-grown pigweeds or kochia weeds to observe compaction effect on roots. You will be able to see if roots are becoming restricted at certain depths or if they are able to grow deep into the soil (Figure 6). A compacted soil layer feels denser than the layers above, and the compacted soil structure appears to be in layers rather than crumbs.
Figure 6. Compaction layer limiting root growth.
A more quantitative way to detect a compacted layer is by using a soil penetrometer (compaction meter). Penetrometers use a metal probe with a cone-shaped tip to measure the force required to push through the soil (Figure 7). This force may be expressed in megapascals (MPa) or pounds per square inch (psi). A reading of over 300 psi (2 MPa) is regarded as too hard for most agricultural roots to penetrate (Aase et al., 2001). As the soil dries out, the compaction reading normally increases, so it is important to conduct compaction measurements when the soil is at field capacity, i.e., soil moisture level after gravity has drained excess soil water from recent irrigation or precipitation.
Figure 7. A soil penetrometer or compaction meter.
Sandy soils reach field capacity about two days after a major rainfall or irrigation event that thoroughly soaked the soil profile. In heavier soils, you may need to wait up to four days before the soil reaches field capacity. It is important when using a penetrometer to note the extent of resistance, the depth at which the compacted layer starts within the soil, and the depth at which you break through the compacted layer. In some cases, you may be able to break through the layer, but in other cases the compacted layer may continue into the subsoil.
Another precaution in using a penetrometer is to make sure that you take many measurements in a given field to be able to reliably estimate the depth at which the compaction is located.
Within a uniform acre, take at least 20 measurements and calculate the average of the measurements. You can take separate measurements for different parts of the field if they have different textures or have been managed differently. The goal is to conduct the measurements such that you capture where the compacted layer is located within the soil for a given field.
Management Strategies
Managing a compacted soil layer will depend on whether the compaction is at the surface or beneath the soil.
Mitigating Surface Compaction
Surface compaction or crusting often results because the aggregates at the soil surface are weak and unprotected from external forces such as rainfall or running water. Managing the soil to increase the organic matter content will go a long way to prevent surface crusting and make soil aggregates more resistant to the impact of rainfall or running water. In cases where a crop has been planted and the crusted surface will impede emergence, shallow cultivation to break the crust will improve crop emergence. A rotary hoe can also be used to break surface crusts even after crop emergence. In some cases, applying irrigation water to soften the soil can improve seedling emergence. Also, mulching the soil surface to prevent a rapid drying down will help delay or prevent crust formation during the period of seedling emergence. Standing stubble can reduce wind reaching the soil surface and slow down soil drying, thus minimizing the incidence of surface crusts.
Avoiding Subsurface Compaction
Subsurface compaction is a more common form of compaction due to modern farming operations that rely on heavy equipment, implements, and tractors. Still, there are several ways to avoid soil compaction under such conditions. Some better management practices that can prevent subsurface soil compaction are:
- Avoid soil tillage operations when the soil is wet. Tilling when the soil is wet leads to a quicker breakdown of soil structure. When soil structure breaks down, soil particles become reconsolidated and are arranged closer to each other to form a compaction layer. This layer will then restrict root and water movement in the soil. The earliest time to get into the field after a rainfall or irrigation event depends on the soil type. Fields with sandy soil, where drainage is fast, can be re-entered without damaging soil structure sooner after rain or irrigation than fields with heavy soils where drainage is slow. Soil structural breakdown due to applied force is greatest when the soil water is close to field capacity or wetter (Figure 8). It is therefore advisable to stay off the field when the soil is very wet to avoid compaction.
Figure 8. Soil compaction under different soil moisture conditions (Adapted from Soehne, 1958). - Practice controlled traffic on the farm. It is best to establish permanent travel lanes for farm traffic. Indiscriminate driving of tractors and other farm machines across the field can lead to compaction of many sections of the field. Establishing paths for movement requires planning and discipline, but will eventually lead to less compaction of the field (Figure 9). Controlling traffic has been shown to lead to higher yields in agricultural soils (Williford, 1980). Depending on your farm operation, you can manually mark the field or use GPS guidance systems to know where traffic paths are located. Cultural practices, such as planting, spraying, and harvesting, can then be done with farm traffic traveling only along established paths.
Figure 9. Practicing controlled traffic will reduce compaction in the field. - Use tractors with the correct type of tires and tire pressures. Tractor tires distribute forces into the soil, which can initiate the compaction process. Therefore, it is essential to use the correct types of tires and inflate them to appropriate pressures to avoid field compaction. Larger tires are better than smaller, skinny tires for spreading out the tractor's weight. Dual tires (Figure 10) have been shown to have a lower compaction effect in the field compared to single-track tires (Arvidsson et al., 2011). Tractor weight is divided among the tires, so extra tires in duals and triples reduce weight on individual tires compared to the single-track tractors. Also, in dual and triple tires, the carrying load on each tire is reduced, thereby reducing the air pressure needed for each tire. The lower tire pressure in duals and triples decreases the depth and intensity of the compaction.
It is also a good practice to regularly check the tire pressure to make sure it is set at the recommended rate. Inflating tires to higher than recommended rates has been shown to increase compaction and lead to relatively less tire traction (Raper et al., 1995). Raper et al. (1995) also showed that the tire shape changed with inflation pressure, with the lower pressure creating a larger footprint that promoted less compaction. The higher inflation pressure caused greater soil compaction about one foot below the soil surface.
Figure 10. A tractor with dual tires. - Reduced tillage practices. Reduced tillage practices can minimize the incidences of soil compaction in agricultural fields by reducing the farm traffic and/or number of passes. Since the soil is not receiving the full range of tillage operations as in the conventional practice, soil quality is improved over time, and the soil organic matter is built up, which minimizes the impact of soil compaction in agricultural fields (Soane, 1988).
- Managing the soil organic matter. Organic matter makes the soil more resilient to soil compaction (Ohu et al., 1986; Soane, 1990) by making the soil more elastic, thus limiting the ability of an applied load to compact the soil. Organic matter also leads to better and stronger soil aggregates that can help the soil withstand compaction pressure (Ekwue and Stone, 1995). It has been shown that soils high in organic matter have higher bearing capacity and are able to withstand farm traffic with less compaction compared to a similar soil with low organic matter. Therefore, management practices that add soil organic matter will make the soil more resilient to surface and subsurface compactions. (For more information on managing soils for increased organic matter, see NMSU Cooperative Extension Guide A-148, Understanding Soil Health for Production Agriculture in New Mexico, available at pubs.nmsu.edu/_a/A148.pdf
Mitigating Subsurface Compaction
Breaking subsurface compaction layer with a subsoiler
Subsurface compaction can limit root growth and prevent adequate growth and yield of crops. Therefore, in many cases where compaction occurs at a reasonable depth, it is possible to use a deep tillage tool like a subsoiler or interrow ripper (Figure 11) to break up the compaction so that roots of crops can grow deeper into the soil. Subsoiling also improves the infiltration rate of the soil, allowing more water to enter into the soil profile.
Fig. 11: Photograph of a subsoiler (A) and Photo of a field where subsoiling was recently performed (B).
Before subsoiling, it is important to know the depth and extent of the compaction layer. Typically, the shank of the subsoiler is set to a depth just below the compaction layer. For example, if there is compaction between 9 and 12 inches, the subsoiler can be set to about 13 to 14 inches to break the compaction layer. Subsoiling is an energy-intensive operation; therefore, going deeper than necessary with the shanks will waste energy. In addition, an appropriately sized tractor should be used to pull the subsoiler, especially in heavy soils.
Using deep-rooted cover crops
Some crops are capable of penetrating compact layers within the soil. These crops could be annual or perennials. In cases where the land does not need to be used immediately, such crops can be planted to overcome the compaction problem. Raper et al. (2000) found that a rye cover crop was the largest single factor for increasing seed cotton yields in a compacted soil, with positive results seen in three of the four years of study. Cover crops have been shown to reduce penetration resistance and bulk density of compacted soils (Ess et al., 1998; Raper et al., 2000). An added advantage of crop-based mitigation strategies is that they add organic matter to the soil, which will lead to better soil structure in addition to breaking up the compaction layer. Examples of crops that can be used to break compacted soil layers include forage radish, annual ryegrass, canola, sunflower, sorghum-sudan, and turnip. Some studies have demonstrated the efficacy of forage radish (Figure 12) in overcoming soil compaction (Williams and Weil, 2004). Similarly, rotations where crops with shallow rooting follow crops with deeper rooting systems or rotating annuals with perennials (for example, alfalfa after annual vegetables) is another tool for managing soil compaction and improving soil health.
Figure 12. Forage radish roots breaking compaction layer in the soil.
Conclusion
Soil compaction can be a great challenge in agricultural fields and can lead to yield reductions depending on the type of crop grown. However, there are many strategies that producers can use to avoid or address soil compaction issues on the farm. Compaction, in many cases, occurs with heavy traffic or when equipment is operated on wet soils. To avoid compaction, it is critical to stay off wet fields until they are dry enough for operating farm machines and equipment. Taking measurements with a penetrometer can provide information about the depth and extent of soil compaction, and this can assist in developing management options.
References
Aase, J., D. Bjorneberg, and R. Sojka. 2001. Zone-subsoiling relationships to bulk density and cone index on a furrow-irrigated soil. Transactions of the ASAE, 44, 577—583.
Alblas, J., F. Wanink, J. Vandenakker, and H.M.G. Vanderwerf. 1994. Impact of traffic-induced compaction of sandy soils on the yield of silage maize in the Netherlands. Soil & Tillage Research, 29, 157—165.
Ankeny, M.D., T.C. Kaspar, and R. Horton. 1990. Characterization of tillage and traffic effects on unconfined infiltration measurements. Soil Science Society of America Journal, 54, 837—840.
Arvidsson, J., and I. Hakansson. 1991. A model for estimating crop yield losses caused by soil compaction. Soil & Tillage Research, 20, 319—332.
Arvidsson, J., H. Westlin, T. Keller, and M. Gilbertsson. 2011. Rubber track systems for conventional tractors—Effects on soil compaction and traction. Soil & Tillage Research, 117, 103—109.
Baumhardt, R.L., R.C. Schwartz, J.C. MacDonald, and J.A. Tolk. 2011. Tillage and cattle grazing effects on soil properties and grain yields in a dryland wheat—sorghum—fallow rotation. Agronomy Journal, 103, 914—922.
Ekwue, E.I., and R.J. Stone. 1995. Organic-matter effects on the strength properties of compacted agricultural soils. Transactions of the ASAE, 38, 357—365.
Ess, D.R., D.H. Vaughan, and J.V. Perumpral. 1998. Crop residue and root effects on soil compaction. Transactions of the ASAE, 41, 1271—1275.
Filipovic, D., S. Husnjak, S. Kosutic, and Z. Gospodaric. 2006. Effects of tillage systems on compaction and crop yield of Albic Luvisol in Croatia. Journal of Terramechanics, 43, 177—189.
Hanna M., and M.M. Al-Kaisi. 2009. Understanding and managing soil compaction [Agronomy Pub 8-1]. Ames: Iowa State University Extension.
Luxmoore, R.J. 1981. Micro-, meso-, and macroporosity of soil. Soil Science Society of America Journal, 45, 671—672.
Morin, J., Y. Benyamini, and A. Michaeli. 1981. The effect of raindrop impact on the dynamics of soil surface crusting and water movement in the profile. Journal of Hydrology, 52, 321—335.
Ohu, J.O., G.S.V. Raghavan, and E. Mckyes. 1986. Effect of reduction of soil compaction through organic-matter incorporation on crop yield. Soil & Tillage Research, 8, 325—325.
Raghavan, G.S.V., E. Mckyes, G. Gendron, B. Borglum, and H.H. Le. 1978. Effects of soil compaction on development and yield of corn (maize). Canadian Journal of Plant Science, 58, 435—443.
Raper, R.L., A.C. Bailey, E.C. Burt, T.R. Way, and P. Liberati. 1995. The effects of reduced inflation pressure on soil-tire interface stresses and soil strength. Journal of Terramechanics, 32, 43—51.
Raper, R.L., D.W. Reeves, C.H. Burmester, and E.B. Schwab. 2000. Tillage depth, tillage timing, and cover crop effects on cotton yield, soil strength, and tillage energy requirements. Applied Engineering in Agriculture, 16, 379—385.
Soane, B., 1990. The role of organic matter in soil compactibility: A review of some practical aspects. Soil & Tillage Research, 16, 179—201.
Soane, B.D., 1988. The role of organic-matter in soil compaction. Journal of the Science of Food and Agriculture, 45, 134—135.
Sohne, W. 1958. Fundamentals of pressure distribution and soil compaction under tractor tires. Agricultural Engineering, 39(5), 290.
Williams, S.M., and R.R. Weil. 2004. Crop cover root channels may alleviate soil compaction effects on soybean crop. Soil Science Society of America Journal, 68, 1403—1409.
Williford, J., 1980. A controlled-traffic system for cotton production. Transactions of the ASAE, 23, 65—70.
Yamulki, S., and S.C. Jarvis. 2002. Short-term effects of tillage and compaction on nitrous oxide, nitric oxide, nitrogen dioxide, methane and carbon dioxide fluxes from grassland. Biology and Fertility of Soils, 36, 224—231.
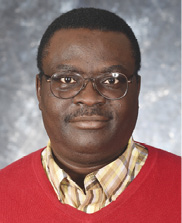
John Idowu is an Extension Agronomist in the Department of Extension Plant Sciences at NMSU. He earned his master's in agronomy from the University of Gottingen in Germany and his Ph.D. in land management from Cranfield University in the UK. His research and Extension activities are focused on sustainable crop production and soil management in New Mexico.
To find more resources for your business, home, or family, visit the College of Agricultural, Consumer and Environmental Sciences on the World Wide Web at pubs.nmsu.edu
Contents of publications may be freely reproduced for educational purposes. All other rights reserved. For permission to use publications for other purposes, contact pubs@nmsu.edu or the authors listed on the publication.
New Mexico State University is an equal opportunity/affirmative action employer and educator. NMSU and the U.S. Department of Agriculture cooperating.
November 2013, Las Cruces, NM